Sepsis is a complex syndrome of concurrent pathophysiological processes [1]. Its incidence is increasing worldwide; therefore, it is essential to understand its pathophysiology correctly [2–6].
Among the pathophysiological processes that develop simultaneously in sepsis, mitochondrial dysfunction and reactive oxygen species (ROS) production play an essential role [7]. ROS are a group of molecules that include oxygen radicals and non- radical oxidizing agents that they are readily converted into radicals [1]. In addition, there are reactive nitrogen species, both radical and non-radical [7, 8]. Several definitions of oxidative stress exist, but the most common one is the imbalance between ROS production and the cellular antioxidant capacity, which potentially damages cells and destroys tissues [9].
Another pathophysiological process described in sepsis is apoptosis, a highly regulated and conserved cell death mechanism in which the cell self-destructs [10]. It is the usual method in which multicellular organisms eliminate undesired or superfluous cells, neutralizing the potential damage caused by cells with defective DNA [10]. Seve-ral intracellular and extracellular triggers activate a cascade of enzymes, the final effectors of which are proteolytic enzymes that cleavage at the cysteine level [10]. Cells in which this process is triggered shrink, condense, and fragment, releasing membrane-bound apoptotic bodies that are gene-rally taken up by other cells. Also, the nucleus is condensed, the DNA is fragmented, and the cellular constituents are not released into the extracellular environment where they could have harmful effects on the surrounding cells, unlike what happens with cell death due to necrosis [10].
In recent years, interest in apoptosis in sepsis has increased. Some studies have shown that apoptosis levels are increased in different models of sepsis [11–14]. Remarkable studies have shown that apoptosis levels are increased in different models of sepsis [11–14]. In other publications, the degree of cell death is disproportionately low compared with the degree of severity of clinical or biochemical presentation of multiple organ failure (MOF) [15]. On the other hand, some authors showed that apoptosis markers could be used as prognostic markers [16–18].
Succinate is a Krebs cycle intermediate that is oxydized in mitochondrial complex II. Different studies have describe that succinate improved oxygen consumption in septic rat muscle [19], prolonged survival [20], and improved liver metabolic profile [21]. In previous studies, it has been shown that succinate reduces ROS levels in septic rats [22].
Both pathophysiological processes (ROS production and apoptosis) and others are the cause of the MOF observed in sepsis. This study aims to observe whether both markers are correlated and, thus, infer if the processes are simultaneous, what their relationship is, and, in addition, probe their association with organ failure.
METHODS
Animals
Male Sprague Dawley rats weighing 200 g, adapted to 12 h light cycle for 7 days, and fed ad libitum at standard temperatures (24°C) were used. The current research was approved by the Institutional Animal Care and Use Committee (CICUAL) of the Faculty of Medicine of the University of Buenos Aires (EXP-UBA: 02282/2012).
There were 4 groups, with 10 rats per group:
Group 1 (Control) did not undergo any intervention during the study.
Group 2 (Succinate) intraperitoneal succinate was administered 2 h before the surgery (which occurred in groups III and IV) and 2 h before the acquisition of the sample.
Group 3 (Sepsis group) underwent caecal ligation and puncture, as described in the corresponding section. Resuscitation was performed with 20 mL kg–1 of NaCl 0.9%, and antibiotic treatment with ceftriaxone (30 mg kg–1) and clindamycin (25 mg kg–1).
Group 4 (Sepsis + Succinate group) in which sepsis was induced as described and which succinate (5 mmol kg–1) was administered 2 h before surgery and 2 h before the sample taking. In addition, resuscitation was performed with 20 mL kg–1 of NaCl 0.9%, and antibiotic treatment with ceftriaxone (30 mg kg–1) and clindamycin (25 mg kg–1).
Between the surgery and the sample taking 24 h elapsed. The study is outlined in Figure 1.
FIGURE 1
Timeline of the succinate experiment in septic rats. The different steps of the experiment are represented. 4 groups were formed as described in Material and Methods: 1 – control, 2 – succinate, 3 – sepsis, 4 – sepsis + succinate. In groups 2 and 4, 5 mmol kg–1 of intraperitoneal succinate were administered with a 0.4 M solution

Caecal ligation and puncture
The procedure was performed under anaesthesia with 100 mg kg–1 of intraperitoneal ketamine and 2.5 mg kg–1 of intraperitoneal xylazine. Using the traditional technique [23–28], skin and aponeurosis incisions, and a midline laparotomy were performed. A plane-by-plane dissection was performed, and once the abdomen was entered, the cecum was identified, and 1 cm was ligated. Then, both sides of the ligated cecum were punctured with a 25 × 8 needle, and, subsequently, a layered suture was performed.
Succinate
5 mmol kg–1 of succinate, from a 0.4 M solution were administered intraperitoneally, as specified above. The solution was prepared with succinic acid (Sigma Chemical Co., St. Louis, MO, USA), adjusted to pH 7.4 with NaOH, and filter sterilized with a 0.2 μm filter (Minisart®, Sartorius, Gottingen, Germany) in a laminar flow cabinet. The chosen dose corresponds to that described in the literature [29].
Serum sample taking and euthanasia
A blood sample was taken through cardiac puncture 24 hours after the surgery of the respective groups. This process was done under anaesthesia with 100 mg kg–1 of intraperitoneal ketamine and 2.5 mg kg–1 of intraperitoneal xylazine. Later, the animal was euthanized by sectioning the great vessels and removing the heart. Blood was centrifuged in dry tubes at 3000 rpm. Then, the serum was separated and frozen at –75°C. The measurements were made within 60 days of the sample taking.
Determination of serum biochemical variables
Levels of creatinine, urea, total bilirubin, and lactic acid were determined in mg dL–1 and processed on a Vitros 5600 (Ortho Clinical Diagnostics, Raritan, NJ, USA) analytical platform, using the dry chemistry method.
Measurement of serum ROS
The measurement of serum ROS was obtained using dichlorofluorescin-diacetate (DCFH). 12 µl of serum were incubated for 10 minutes in 1000 µl of TE buffer solution, and 10 µl of NaOH were added to separate the diacetate and thus activate the dichlorofluorescin. The emitted fluorescence was measured with Jasco FP770 (Jasco, Budapest, Hungary) equipment. An emission spectrum between 500 and 550 nm was used with each sample. The expressed value is that of the emission at 525 nm because this is the emission peak of DCFH. This fluorophore (dichlorofluorescin diacetate) needs the diacetate to be separated, activated, and emit a signal at 525 nm. This step takes place within the cells due to the presence of esterases. This technique is also described for measurements in extracellular fluids [30–33]. In this case, it is necessary to previously separate the diacetate through prior incubation with NaOH, without the need for estera-ses [30–33].
Determination of serum caspase-3
This measurement was taken using the ELISA technique, with a MyBioSource Rat Caspase 3 ELISA Kit (Catalogue #MBS763727 Lot #R0143D032), following the manufacturer’s specifications. The animal serum was used as described above. Samples were measured in an EMP M201 Microplate Reader (Shenzhen Emperor Electronic Technology Co., Ltd.).
Statistical analysis
The mean with 95% confidence interval (95% CI) was used for all results. Shapiro-Wilk test for normality was used, and all variables had normal distribution with a W value between 0 and 1 and a P-value higher than 0.05. To analyse whether there were significant differences in continuous variables between 2 groups the Student's t-test was used, and among 3 or more groups the ANOVA test was used. The Pearson or Spearman tests were used to correlate 2 variables. In all cases, a statistical significance of P < 0.05 was assumed. Statistical analyses were performed using: EPIinfo 7.0, Statistix 7.0, and Graph Pad Prism 8.0.2.
RESULTS
The mean and confidence interval of serum ROS levels, serum caspase-3 levels, serum creatinine, and total bilirubin levels are expressed in Table 1 along with the result of the ANOVA test.
TABLE 1
Serum levels of biochemical determinations. Results from serum measurements of the 4 groups of rats. ANOVA test was used to find differences between groups. The P-value column expresses the results of the ANOVA test, comparing the 4 groups. All values are represented as mean (95% CI)
Significant differences were found between serum ROS levels measured with the DCFH technique (P = 0.017). However, the levels of serum caspase-3, serum creatinine, and total bilirubin did not differ significantly.
Differences between groups in ROS levels were analysed (Figure 2). There were no significant differences in ROS levels between the control group and the succinate group (P = 0.29). Also, there were no differences between the control group and the sepsis + succinate group (P = 0.18). On the other hand, there were significant differences between the control group and the sepsis group (P = 0.012). Furthermore, the administration of succinate reduced ROS levels in the sepsis + succinate group compared with the sepsis group showed statistical significance (P = 0.004).
FIGURE 2
ROS levels in response to succinate administration. The mean values and confidence interval of ROS (DCFH emission at 525 nm) are expressed in the 4 groups as described in Material and Methods. There were significant differences between the control group and the sepsis group (P = 0.012) (*), and between the sepsis group and the sepsis + succinate group (P = 0.004) (#)
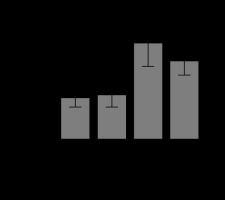
Also, differences between groups in serum caspase-3 were analysed (Figure 3). There were no significant differences between the control group and the succinate group (P = 0.26), the sepsis group (P = 0.15), and the sepsis + succinate group (P = 0.24). Additionally, there were no significant differences between the sepsis group and the sepsis + succinate group (P = 0.39) regarding serum levels of caspase-3.
FIGURE 3
Levels of activated serum caspase-3 in response to succinate administration. The mean values and their confidence interval of serum activated caspase-3 are expressed in the 4 groups as described in Material and Methods. There were no significant differences among the groups
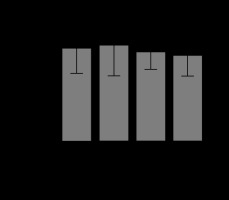
In creatinine levels, there were no significant differences between the control group and the succinate group (P = 0.44), between the control group and the sepsis group (P = 0.1), or between the sepsis group and the sepsis + succinate group (P = 0.14). However, a significant increase in creatinine levels was observed between the control group and the sepsis + succinate group (P = 0.009) (Figure 4). Lastly, in total bilirubin levels, there were no significant differences between the control group and the succinate group (P = 0.59), nor between the control group and the sepsis + succinate group (P = 0.21). Furthermore, there were no differences between the control group and the sepsis group (P = 0.49), nor between the sepsis group and the sepsis + succinate group (P = 0.67) (Figure 5).
FIGURE 4
Serum creatinine levels in response to succinate administration. The mean values and their confidence interval of serum creatinine are expressed in the 4 groups as described in Material and Methods. There were differences between control and sepsis + succinate group
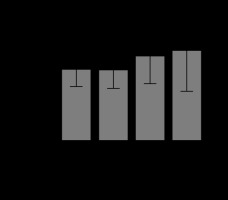
FIGURE 5
Total bilirubin levels in response to succinate administration. The mean values and their standard deviation of total serum bilirubin are expressed in the 4 groups as described in Material and Methods. There were no significant differences among the groups
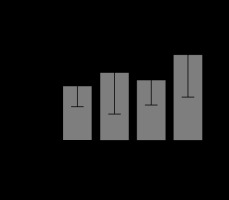
The Pearson test was used to analyse the correlation between serum ROS and caspase-3 levels in animals in all 4 groups. There was no correlation between the levels of both variables. A scatter plot was made with the values of both variables (R = –0.17; P = 0.3) (Figure 6). There was no correlation when analysing only the groups that did not receive succinate (R = –0.16; P = 0.5), nor the groups that received succinate (R = –0.25; P = 0.32).
FIGURE 6
Correlation between systemic ROS levels and serum caspase-3. There was no correlation between both variables (R = –0.17, P = 0.3). Emission values are expressed at 525 nm DCFH as a ROS marker
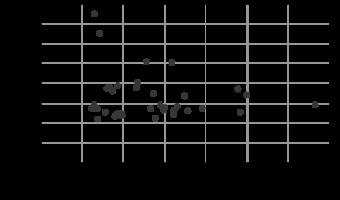
No correlation was found among ROS and creatinine (R = 0.06; P = 0.71), urea (R = –0.24; P = 0.15), and total bilirubin (R = 0.16; P = 0.33). Also, no correlation was found between serum caspase-3 levels and creatinine concentration (R = 0.056; P = 0.74), urea (R = 0.15; P = 0.37), and total bilirubin (R = 0.17; P = 0.31).
DISCUSSION
The aim of the administration of succinate to the rats was to provide a substrate for complex II of the electron transport chain and reducing ROS production. It is known that oxidative stress can trigger apoptosis in different pathophysiological models [9, 10]. Therefore, the rat caecal puncture model was used as a sepsis model, and succinate was administered as a means of controlling ROS production and assessing whether this had any effect on the levels of apoptosis and sepsis-induced organ failure. Likewise, it was analysed if there was a correlation between ROS and apoptosis to evaluate if both are related, in order to establish a relationship between both processes.
It was determined that systemic ROS levels are elevated in septic rats when compared with the control group and that the administration of parenteral succinate reduces the production of these species in septic rats. In contrast, parenteral succinate administered in rats that did not undergo caecal ligation and puncture does not cause any changes regarding the control group, suggesting that succinate does not affect non-septic rats. In previous studies, it has been documented that succinate reduces serum ROS levels, but it does not improve creatinine levels in septic rats [22].
There are contradicting publications regarding succinate levels in pathological situations, and some of them regard hypoxia-reoxygenation. Chouchani et al. [34] demonstrated an accumulation of succinate during hypoxia-reoxygenation cycles in mice, though various tests showed that glucose, palmitate, glutamate, and GABA do not contribute to its accumulation. Instead, the cause of the accumulation is fumarate. On the other hand, Wijemars et al. [35] analysed biopsies of transplanted kidneys and observed a drop in tissue succinate accumulation. However, hypoxia-reoxygenation is not the only pathophysiological mechanism in sepsis.
Several studies have shown changes in the processes that occur within the mitochondria during sepsis, among which the electron transport chain is affected. The literature is inconclusive regarding the activity rank of the different complexes at this level. Lorente et al. [36] demonstrated decreased action in the electron transport chain in complex IV, while Brealey et al. [37–39] found decreased complex I activity but found no differences in complexes II, III, or IV. Furthermore, the same group demonstrated that the activity of complexes II and III remained unchanged both in the muscles and the liver in septic rats [37–39]. Conversely, in those organs, complex I activity seem-ed to increase with the severity of sepsis in rats.
In another study done on septic rats, using the cecum ligation and puncture technique, the infusion of dimethyl succinate improved the survival of the rats [20]. On the other hand, it was demonstrated that, in rats that were administered LPS, dimethyl succinate infusion improved ATP levels and the ATP/ADP ratio, which would suggest a recovery in the activity of the electron transport chain [21]. A different study determined that complex I acti-vity was decreased in the soleus muscle of rats with moderate/severe sepsis caused by the intraperitoneal administration of stool preparation. In contrast, there were no changes in complex II activity in control group rats. In addition, the administration of malate and glutamate (as complex I substrates) and succinate (as complex II substrate) improved muscle oxygen consumption compared with the administration of malate and glutamate alone. Furthermore, this improvement was more pronounced in rats with moderate/severe sepsis than in rats with mild sepsis [19].
Caspase-3 was chosen as an apoptosis marker because it is commonly used in the literature [12, 40–43], and its serum levels were used to determine apoptosis levels [44]. The aim of measuring serum caspase-3 was to determine the extent of apoptosis in tissues.
There were no differences in serum caspase-3 levels among the 4 groups, nor in tissue caspase-3 levels in the liver and the kidney among the 4 groups. It is known that, in animal models of sepsis, apoptosis levels in different organs are increased. For example, in a study done on rats exposed to LPS, it was observed that the activity of caspases-3, -8, and -9, as well as TNF-a levels, increased in the left ventricle [45]. On the other hand, rats that underwent caecal ligation and puncture and were subsequently euthanized at different times showed an increase in renal apoptosis, measured using the TUNNEL technique, with a peak at 6 hours. Furthermore, renal apoptosis measured by cytokeratin 18 fragment M30 had 2 peaks, one at 6 hours and another at 48 hours [46]. In the study, the determination of markers was done at 24 hours and 48 hours, respectively. The mortality of the rats was very high and could have altered the results and, at 6 hours, it could be an early measurement, and there probably would have been no differences in serum ROS levels. Therefore, the difference between the results obtained in this research and those obtained in the above-mentioned publications could be due to the kinetics of elevation in serum of those markers.
On the other hand, immunohistochemical studies show that, in sepsis, there is a higher degree of lymphocyte and digestive tract cell death, whereas, in the kidney, liver, and lungs, cell death is lower [42, 47, 48]. In addition, in a study done on sheep (with E. coli infusion), it was observed that there was no increase in apoptosis markers in the kidney in septic animals. However, in animals that recovered from sepsis (the infusion of bacteria was suspended, and gentamicin was administered) levels of those markers increased in the kidney [50]. These findings coincide with the results presented in this study because the animals were septic when taking the sample.
In addition, there was no correlation between serum caspase-3 levels and serum ROS levels. In a previous work with septic patients, we showed that septic patients have higher levels of caspase-3 but not higher levels of serum ROS, and both markers did not correlate [51]. The conclusion could be that both processes are not related or do not co-occur in sepsis.
Creatinine levels increased by 22% in septic rats, but the differences were not statistically significant compared with the control group. In contrast, serum creatinine levels in septic rats with parenteral succinate did not decrease compared with septic rats without treatment, as it was seen in DCFH levels. Also, the sepsis + succinate group had higher creatinine levels than control group. Creatinine is a late marker of kidney failure, and a marker such as NGAL, which would give an early account of the damage and show significant differences among the different groups, was not used [52–54]. This marker could be useful to understand the kidney function in the different groups.
Lastly, there was no correlation between ROS levels and creatinine, total bilirubin, and urea levels. This observation suggests that there would be no pathophysiological association between ROS levels and organ failure. This is like previous studies in which there was no correlation between ROS levels and serum creatinine levels [22]. In this study, several of the rats used were also included in the previous study to improve the use of resources and reduce the number of rats for animal ethics issues. As previously stated, the cause and pathophysiology of MOF is multifactorial, which is probably one of the causes for the lack of correlation among the variables mentioned above. That is to say that the increase in creatinine levels would be determined by ROS status and other previously described pathophysiological mechanisms, such as pH, coagulation, endothelial, and microcirculation alterations, among others.
Total bilirubin levels in non-septic rats did not increase with succinate administration compared with the control group. In addition, there were no differences in total bilirubin levels in septic rats with and without treatment with succinate. The only reference found in the literature regarding the effect of succinate on the liver of septic rats states that succinate infusion improves the concentration of β-hydroxybutyrate, increases ATP concentration in hepatocytes, and glucose oxidation, and decreases the lactate/pyruvate ratio [21].
The main limitations of the study are the single time measurement of ROS and apoptosis markers, which did not allow the detection of a potential asynchronic correlation between them. The other limitation is that renal function was assessed with creatinine but not with N-GAL. The main strength is that serum ROS were measured and not oxidative stress, and it was correlated with apoptosis markers, and with kidney and liver damage markers.
CONCLUSIONS
Succinate reduces ROS levels in septic rats but does not reduce caspase-3 levels as an apoptosis marker. Also, there was no correlation between ROS levels and caspase-3 levels, suggesting that both processes are not connected. Finally, succinate did not reduce creatinine and bilirubin levels as markers of organ failure, and there was no correlation between the apoptosis marker and serum ROS measurement and organ failure markers.