In 2020, approximately 19.1 million deaths were attributed to cardiovascular disease (CVD) globally, and CVD remains the leading cause of human morbidity and mortality [1]. The American Heart Association (AHH) reported that 840,768 individuals died due to CAD in the United States of America in 2016 [2]. According to the AHH, atrial fibrillation (AF) was the underlying cause of death in 24,855 people in 2016 [3]. The average annual direct and indirect cost of CVD and stroke in the United States was an estimated $351.2 billion from 2014 to 2015 [2].
Given the high burden of CVD on public health and costs for the health system, further refinement of cardiovascular risk stratification is warranted. Interest in epicardial adipose tissue (EAT) as a potential biomarker for major adverse cardiac events (MACE) has risen in the medical community. Although EAT only represents a small fraction of the total visceral adipose tissue (VAT), EAT significantly impacts the heart due to its unique anatomic relationship with the adjacent myocardium [4]. Pro-inflammatory cytokines released by the EAT cells can directly interact with the myocardium via paracrine and vasocrine pathways, as EAT and the adjacent myocardium share the same microcirculation in the absence of a fascial barrier between the myocardium and EAT [4, 5].
EAT is a storage depot for free fatty acids (FFA). Thus, EAT may protect the heart against toxic FFA levels. It has also been suggested that EAT serves as a buffer for the arterial circulating FFAs. Therefore, EAT may be considered a local energy source of FFA during times of high energy demand by channelling fatty acids to the myocardium [6, 7].
EAT can be assessed using cardiac computed tomography (CCT), echocardiography, and cardiac magnetic resonance (CMR) [8–10]. Authors have advocated the integration of biomarkers, such as EAT volume, into routine reporting of coronary artery calcium (CAC) scoring CT to enhance cardiovascular risk stratification [11].
In this review, we discuss the physiologic and anatomical features of EAT (Section 1), the advantages of the several cardiac imaging modalities (Section 2), and the ability of EAT to predict MACE (Section 3). We focus on the prognostic role of EAT in coronary artery disease (CAD), post-procedural atrial fibrillation, valvular heart disease, left ventricular (LV) remodelling, heart failure, and critically ill patients admitted to the intensive care unit (ICU).
SECTION 1: PHYSIOLOGICAL AND ANATOMICAL FEATURES OF EAT
Special anatomical relationship between EAT and the myocardium
The adipose tissue of the heart consists of epicardial adipose tissue (EAT) and pericardial adipose tissue (PAT). EAT is situated between the visceral pericardium and the myocardium. PAT is adjacent to the external surface of the parietal pericardium, and thus outside the visceral pericardium [4, 5, 12]. EAT and PAT have different embryologic origins. The epicardium harbours a population of mesothelial cells originating from the septum transversum [4, 5, 12]. EAT originates from the splanchnopleuric mesoderm, while PAT originates from the primitive thoracic mesenchyme [5, 12]. EAT and PAT have different and distinct vascularization. EAT is vascula-rised by branches of the coronary arteries. PAT has a non-coronary vascular supply [5, 12].
EAT represents approximately 20% of the heart mass in healthy lean persons, commonly found in the interventricular and atrioventricular grooves. Sometimes, the fat accumulation extends from the epicardial surface into the myocardium [13].
From a physiological point of view, the principal difference between EAT and other visceral adipocyte tissue depots is the absence of a muscle fascia that divides the EAT and the myocardium. Thus both structures share the same microcirculation [4, 14]. Due to its anatomical proximity to the myocardium without fascial barriers, EAT can interact locally with the myocardium by vasocrine or paracrine secretion of pro-inflammatory adipokines [4, 15, 16]. Animal experiments have demonstrated that periadventitial application of that bio-active molecules promotes influx of inflammatory cells into the arterial wall and induces intimal lesions [17–19]. This process was called an “outside-to-inside” mechanism by Krishnan et al. [20].
Company et al. have suggested a further anatomical distinction between EAT myocardial and pericoronary epicardial fat [21]. Pericoronary epicardial adipose tissue (PCAT) is located directly around the coronary artery adventitia, whereas myocardial epicardial fat is located directly over the myocardium without any layer separating them. The PCAT CT attenuation is significantly increased across the culprit lesion precursor in patients with acute coronary syndrome (ACS), compared to non-culprit lesions of these patients and to lesions of patients with stable CAD [22]. Spontaneous coronary artery dissection (SCAD) has been associated with higher PCAT compared with patients without SCAD [23]. Nevertheless, it remains unclear whether the two components are functionally distinct [4].
EAT contains many ganglia and interconnecting nerves [5, 15]. Neuroprotection of the cardiac autonomic ganglia and nerves is a feature attributed to EAT [16]. A direct correlation between increased EAT thickness and cardiac sympathetic denervation in heart failure has been reported [24]. Epicardial fat accumulation independently predicts impaired heart rate recovery in obese patients with obstructive sleep apnea [25]. More research is needed to determine the exact role of EAT in the autonomous nerve regulation of the heart.
There are conflicting data on EAT volume changes with aging [26, 27]. While most autopsy series have failed to demonstrate a correlation between EAT and age, Tansey et al. reported increasing intramyocardial fat infiltration with increasing age [27].
EAT: Histological aspects
Many authors have emphasised that not all adipose tissues (AT) are alike [28–31]. Taking into account that the cytoplasm of adipocyte cells represents approximately 10% of the cell volume, AT is a tissue with high metabolic activity, and the FFA-glucose turnover is faster in VAT than in subcutaneous adipose tissue (SAT) [32, 33].
EAT is a particular visceral adipose depot. EAT adipocytes are smaller than adipocytes in other SAT or VAT depots. Besides providing mechanical protection, EAT also acts as a readily accessible energy reservoir for the myocardium. Animal experiments by Marchington and Pond have generated data supporting this hypothesis [6]. EAT’s high FFA turnover allows direct distribution of FFA by diffusion to the adjacent myocardium [4].
EAT and cytokine secretion
EAT can locally secrete proinflammatory adipokines, as was demonstrated in a study involving humans with severe CAD undergoing elective CABG surgery. Paired samples of EAT and subcutaneous adipose tissues demonstrated that EAT is a source of several inflammatory mediators in high-risk cardiac patients [34]. Many reviewers have suggested that cytokines released by EAT interact with the adjacent myocardium via paracrine and vasocrine pathways, inducing their unfavourable effects on heart function [4, 5, 31, 35] (Figure 1). As mentioned above, there are data from some animal in vivo experiments that periadventitial application of endotoxin, monocyte chemoattractant protein-1 (MCP-1), IL-1β, or oxidised LDL initiates influx of inflammatory cells into the arterial wall and intimal lesions. These studies suggest that bio-active mole-cules released from the pericoronary tissues may modify arterial homeostasis [17–19].
FIGURE 1
The role of epicardial adipose tissue (EAT) in the development of cardiovascular diseases and cardiovascular complications during COVID-19. Increased EAT volume has been associated with increased body mass index (BMI), subcutaneous adipocyte tissue (SAT), sagittal abdominal diameter (SAD) and waist-to-hip ratio (W : H ratio), suggesting a pathophysiological link between EAT and increased intra-abdominal pressure (IAP) [Figure adapted and reproduced with permission from Konwerski et al. [70] under the open access CC BY 4.0 Licence]
AF – atrial fibrillation, Ang 1-7 – angiotensin 1-7, BMI – body mass index, CAD – coronary artery disease, CCL-2, -5, -13 – chemokine ligand-2, -5, -13, CXCL-1 – chemokine ligand 1, FABP4 – fatty acid binding protein 4, GLUT-4 – glucose transporter type 4, HfpEF – heart failure with preserved ejection fraction, IAP – intra-abdominal pressure, IL-1β, -6, -8 – interleukin-1β, -6, -8, RBP4 – retinol binding protein 4, SAD – sagittal abdominal diameter, sPLA2-IIA – secretory phospholipase A2, TNF-α – tumour necrosis factor α, W : H – waist-to-hip ratio
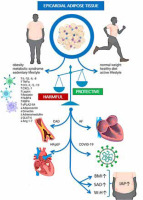
A detailed review of the extensive literature on cytokine release by EAT is outside the scope of this paper. In a review, Guzzardi and Iozzo described the harmful EAT-promoted mechanisms to the heart via inflammation and the role of cytokines therein as a cascade of events [36]. They distinguished four steps, as follows.
First, myocardium exposed to an FFA overflow results in consensual expansion of epicardial adipose tissue [36]. Lipid accumulation in EAT cells causes cell enlargement, subsequently decreasing oxygen delivery. Subsequently, the local hypoxia in combination with FFA overload activates hypoxia-sensitive pathways in adipose tissue with ROS production, activation of JNK1 and IKK/NF-κB pathways, and induction of genes involved in the inflammatory response [37]. This hypoxic and inflammatory status is characterised by increased production and release of proinflammatory cytokines, such as C-reactive protein, TNF-a, and IL-6 [36].
In the next step, these cytokines activate the NF-κB pathway and initiate, via intracellular signals, macrophage and T cell recruitment with tissue infiltration. Most TNF-a and a significant part of IL-6 expression in adipose tissue has been attributed to the macrophages. Finally, they also release other proinflammatory cytokines, inducing a feed-forward cycle, aggravating the inflammatory status and its propagation to the adjacent myocardium via diffusion [36].
A CMR spectroscopy study demonstrated that excessive EAT volume correlates with chronic FFA overload of the myocardium, resulting in intramyocardial fat accumulation. Intramyocardial fat was correlated with FFA levels (r = 0.76; P = 0.017) and EAT volume (r = 0.69; P = 0.042) [38].
Myocardial steatosis was also documented in subjects with CAD, thus suggesting a potential role for myocardial hypoxia in myocardial fat accumulation [39].
Metabolism: the glucose-fatty acid cycle or Randle cycle
The participation of AT in glucose metabolism can be described in terms of the inflow and outflow of hormones and energy substrates at the cellular level [31]. This alternating switch of substrate supply, which has been termed ‘metabolic flexibility’ [40], ensures appropriate energy loading and mobilization following the feeding cycle.
The main biochemical pathway that links AT accumulation to insulin resistance is the glucose-fatty acid cycle or the Randle cycle. Randle et al. [41] demonstrated that adding FFA to the perfused rat heart muscle and diaphragm increased FFA oxidation at the expense of carbohydrate oxidation.
An in vivo study examined the effect of acute physiologic elevations of FFA concentrations on glucose production and uptake in normal subjects under three controlled experimental conditions: euglycaemic hyperinsulinaemia (group A), hyperglycaemic hyperinsulinaemia (group B), hyperglycaemia with relative insulinopenia and hyperglucagonaemia (group C). Under conditions A and B, the percentage inhibition of glucose uptake after FFA administration was positively correlated with the total rate of glucose uptake. In persons in group C, simulating a diabetic state, lipid infusion had no effect on glucose uptake but markedly stimulated endogenous glucose production. Under the same conditions as in group C, a glycerol infusion producing plasma glycerol levels like those achieved with lipid-heparin enhanced endogenous glucose production. The authors conclude that in the well-insulinised state, raised FFA levels effectively compete with glucose for uptake by peripheral tissues, regardless of the presence of hyperglycaemia. On the other hand, if insulin is deficient, elevated rates of lipolysis may contribute to hyperglycaemia not by competition for fuel utilization, but through enhancement of endogenous glucose output [42].
The KANWU study demonstrated that the total amount of fat influences insulin sensitivity only when it exceeds a threshold of 35–40% of daily energy intake. If the threshold is not exceeded, a cri-tical factor in the induction of IR is not the amount of fat itself but its composition, i.e. the types of die-tary FFA [43].
A more recent randomised double-blind crossover study compared three controlled 4-week diets enriched with different fatty acids: a monounsaturated fat diet (M), a saturated fat diet (S), and the trans fatty acid diet (T). The mean insulin sensitivity index (S(I)) decreased by 24% on the S versus M diet in overweight subjects but was unchanged in lean subjects. Insulin secretion was unaffected by diet. Subjects oxidised the least fat on the M diet and the most fat on the T diet. The authors concluded that dietary fatty acid composition significantly influenced fat oxidation but did not impact insulin sensitivity or secretion in lean individuals. Overweight individuals were more susceptible to developing insulin resistance on high-saturated fat diets [44].
Jørgensen et al. examined the effects of early life exposure to either a high fat (HF) or a high sucrose (HS) diet for 12 weeks after weaning compared to rats with a normal diet. They found that rat muscle mitochondria show the normal Randle type fat- carbohydrate interaction (metabolic flexibility) seen in vivo. This regulatory mechanism of carbohydrate-fat metabolic interaction was lost in mitochondria obtained from animals exposed for 12 weeks to a HF or a HS diet as compared to rats given a normal diet. Furthermore, the calculated TCA flux in the isolated mitochondria decreased significantly after the HF diet (~50%) and HS diet (~75%), compared with the chow (a standard high fibre diet containing complex carbohydrates, with fats from a variety of vegetable sources for rodents) group. Thus, it appears that obesogenic diets have a major influence on key metabolic performance of skeletal muscle mitochondria [45].
To test the hypothesis that pyruvate dehydrogenase complex (PDH) links lipid exposure to skeletal muscle insulin resistance through a glucose-fatty acid cycle in which increased fatty acid oxidation increases acetyl-CoA concentrations, and inactivating PDH and decreasing glucose oxidation, Rahimi et al. used a mouse model lacking pyruvate dehydrogenase kinase 2 and 4 (double knockout (DKO)), which results in constitutively activated PDH. Counterintuitively, the increased glucose oxidation in DKO muscle was accompanied by reduced insulin-stimulated muscle glucose uptake. The authors concluded that modulation of oxidative substrate selection to increase muscle glucose utilization surprisingly results in muscle insulin resistance, offering genetic evidence against the glucose-fatty acid cycle hypothesis of muscle insulin resistance [46].
EAT is a storage depot for FFA and can be considered a local energy source of FFA during times of high energy demand by releasing FFA to the myocardium [6, 7].
Besides the Randle cycle, other mechanisms link insulin resistance to obesity. By secretion of pro- inflammatory cytokines, chronic inflammation in adi-pose tissue may contribute to insulin resistance and type 2 diabetes mellitus (T2DM) [47, 48]. Infiltration of hypertrophic adipose depots by macrophages and T-cells may also release inflammatory cytokines in AT [49]. Macrophage infiltration has been associated with insulin resistance [50] (Figure 1).
Role of adipose tissue blood supply
The metabolic activities of AT, such as FFA turnover, require blood supply [51]. Adipose tissue is strongly vascularised, and fat blood flow facilitates the storage and release of lipids. In humans, obesity results in reduced capillarization and cellula-rity of AT [52]. Impaired regulation of AT blood flow is associated with insulin resistance [51]. A recent study found that blood flow and FFA uptake were higher in VAT than in SAT or skeletal muscle in lean individuals, consistent with the higher metabolic activity of VAT [53].
Another study, using positron emission tomography (PET) to quantify both blood flow and glucose uptake during a euglycaemic hyperinsulinaemic clamp, demonstrated a higher tissue-specific blood flow and glucose uptake in intraperitoneal VAT than femoral SAT [54].
Based on a literature review, Camastra and Ferrannini concluded that reduced blood supply is an essential factor impairing the in vivo insulin-mediated glucose uptake in both SAT and VAT [31].
Adipose tissue oxygenation: role of the mitochondria
Adipocytes consume adenosine-tri-phosphate (ATP) to maintain their activities [31]. The role of the mitochondria is pivotal in the physiology of AT, as ATP is generated in the mitochondria [55]. Mitochondrial dysfunction due to nutrient overload is a characteristic of obesity [56]. Reduced mitochondrial function has also been implicated in the aetio-logy of T2DM [57]. A study demonstrated that weight loss by bariatric surgery induced an increase in the number of mitochondria, a reduction of the adipocyte size, and improved insulin sensitivity [58]. Others have suggested that the mitochondrial gene-ration of reactive oxygen species (ROS) may connect mitochondrial dysfunction to insulin resistance by overwhelming the cellular antioxidant capacity, resulting in oxidative stress [59]. AT mass can cause AT hypoxia, leading to systemic insulin resistance, local AT inflammation, and fibrosis in rodents [60]. Human data, studying SAT oxygenation [61] and in vivo AT blood flow [54], demonstrated that fat hypoperfusion and hypoxia contribute to insulin resistance.
EAT and metabolic syndrome, chronic kidney failure, and haemodynamic parameters
The Randle cycle, the mitochondrial function, and the pro-inflammatory properties of EAT mentioned above are the principal pathophysiological links between EAT and metabolic syndrome (MetS).
Sharda et al. observed that EAT thickness positively correlated with fasting blood sugar, LDL cholesterol, triglycerides (Figure 1), total cholesterol, and anthropometric measurements such as BMI, sagittal abdominal diameter and waist circumference, and waist-hip ratio. Furthermore, EAT thickness was more significant in metabolic syndrome cases [62].
Wang et al. reported that CTCA measured EAT thickness in the left atrioventricular groove allows an accurate assessment of metabolic risk, which could not be accounted for by anthropometric indexes and intraabdominal visceral fat [63].
Studies have associated EAT volume with unfavourable clinical outcomes in chronic kidney disease (CKD) [64, 65]. Interestingly, analogously to the way that EAT is an organ-specific adipose tissue impacting the heart, there is growing evidence that the perirenal adipose tissue (PF) has a significant impact on renal pathophysiology [66]. It was demonstrated that PF is an independent predictor for CKD progression and is associated with cardiorenal dysfunction [67].
The association between EAT and haemodynamic variables is not well established. Only a few studies have sought correlations between EAT and invasively measured haemodynamic parameters. A study found that EAT was associated with right ventricular end-diastolic pressure (RVEDP) but not with left-sided filling pressures (pulmonary capillary wedge pressure and LV end-diastolic pressure) [68]. Another study found a modest correlation between EAT volume and pulmonary vascular resistance in Fontan patients [69].
A Medline search, entering the terms ‘epicardial adipose tissue’ and ‘intra-abdominal pressure’ (IAP), revealed no records. Similarly, the Medline search found no studies searching for a correlation between EAT and central venous pressure. Given the association of EAT volume with BMI, W : H ratio, and sagittal abdominal diameter, a pathophysiological link between EAT and intra-abdominal pressure can be postulated (Figure 1).
The association between EAT and heart failure with preserved ejection fraction (HFpEF) has been established [70] and will be discussed in Section 3.
Given the correlation between EAT, BMI, and MetS, is EAT volume an independent predictor of MACE?
In the sections mentioned above, we discussed the correlations between MetS and EAT volume, raising the question whether EAT volume is an independent predictor of MACE. Some large studies have provided some insight.
A study with a median follow-up of 4.7 years included 1030 patients with type 2 diabetes. The composite endpoint was defined as CVD event and mortality [71]. The investigators analysed the results according to 3 models, including the classical CVD risk factors. Analyses were unadjusted (model 1), adjusted for age and sex (model 2), plus systolic blood pressure, body mass index (BMI), low-density lipoprotein (LDL), smoking, diabetes duration, and glycated haemoglobin (HbA1c) (model 3).
Patients with increased EAT (> median level) had increased risk of the composite endpoint in model 1 [hazard ratio (HR): 1.46 (1.13; 1.88), P = 0.004], and borderline in model 3 [HR: 1.32 (0.99; 1.77), P = 0.058]. However, increased EAT was associated with a 41% increased risk of CVD and mortality for men in the robust multivariable model 3 (P = 0.041). The net reclassification index improved when high EAT was added to model 3 (19.6%, P = 0.035) [71].
Another study, including 2068 asymptomatic participants undergoing prospective coronary artery calcium (CAC) scoring by CT in the EISNER study (Early-Identification of Subclinical Atherosclerosis by Non-invasive Imaging Research), demonstrated that persons with MetS had a greater prevalence of non-alcoholic fatty liver disease (NAFLD), increased EAT volume, and lower EAT attenuation compared to those without MetS [11].
At the 14-year follow-up, MACE occurred in 10.8% of the participants. In multivariable Cox regression, MetS was associated with increased risk of MACE (P = 0.01) independently of CAC score, although not after adjustment for EAT measures (P = 0.27). In a separate Cox analysis, NAFLD predicted MACE (HR 1.78 [95% CI 1.21–2.61], P = 0.003) independently of MetS, CAC score, and EAT measures. Adding EAT volume to current risk assessment tools resulted in significant net reclassification improvement for MACE (22% over the atherosclerotic cardiovascular disease (ASCVD) risk score; 17% over the ASCVD risk score plus CAC score) [11].
Overall, these studies seem to confirm the incremental value of EAT, when EAT is added to the classical ASCVD risk factor or CAC score.
SECTION 2: IMAGING MODALITIES MEASURING EAT
Cardiac CT
EAT volume can be assessed on non-contrast-enhanced CT and contrast-enhanced CTCA images [72–74]. Cardiac computed tomography (CCT) allows a clear depiction of the pericardium necessary for volumetric assessment of the EAT volume (Figure 2). Compared to echocardiography and CMR, CCT has the best spatial resolution [75] and visibility [9] of the pericardium, allowing reliable (manual) contouring of the visceral pericardial layer. Subsequently, the CT filter selects the voxels within the visceral pericardium with a CT attenuation coefficient attributed to adipose tissue (range –190 Hounsfield Units [HU] to –30 HU for non-contrast-enhanced CCT) [9, 75]. The total EAT volume is determined by summing all axial slices portraying EAT (Figure 3). EAT volumes can be assessed semi-automatically or by automated algorithms (quantifying both EAT volume and attenuation) [76–79]. Volumetric quantification of EAT by cardiac CT is highly reproducible [78].
FIGURE 2
Example of a non-contrast enhanced (panel a) and a contrast enhanced cardiac CT image (panel B). Note the good visibility of the pericardium (arrows) allowing a clear delineation of the epicardial fat (EAT) and adipocyte tissue outside the visceral epicardium
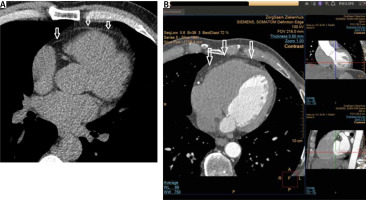
FIGURE 3
Example of cardiac CT acquired EAT volume from non-contrast enhanced images used to determine the Agatston calcium score. Panel a shows an upper axial slice of the heart. Panel B shows an axial slice at the level of the mitral valve. Note the mitral annular calcification. After manual contouring of the pericardium in the axial slice, the software calculates the voxels within the region of interest (ROI) with a CT attenuation coefficient attributed to adipose tissue. In this case, the filter setting defines adipose tissue as the voxels with CT attenuation number ranging from –190 HU to –30 HU (marked in the red ellipse). By summing all axial slices portraying the pericardium, the total EAT volume is calculated and mean EAT radiodensity expressed in HU is calculated (marked in the green ellipse)
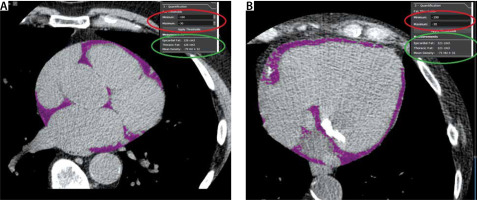
Studies have investigated the influence of contrast enhanced CT, CT tube current, and non ECG- gated chest CT on EAT volume, using 120 kV non- contrast-enhanced cardiac CT as the reference [73, 77]. A study compared two independent cohorts referred for routine CTCA. One cohort received a low-voltage and a standard voltage non-contrast acquisition (120 and 100 kV), while the other cohort underwent non-contrast and contrast-enhanced CT. The lower threshold for EAT was consistently set at –190 HU in all patients. Compared to non-enhanced 120 kV acquisition with an upper threshold of –30 HU as the reference, an upper threshold of –40 HU in the 100 kV population showed the best correlation (r = 0.961, P < 0.05). Significant overestimation was found for upper thresholds of –20 and –30 HU and significant underestimation for –50 HU. In non-contrast vs. contrast-enhanced acquisitions, there was a significant underestimation of EAT volume for contrast-enhanced scans (mean difference 31 mL, 95% limits of agreement 27 to –89 mL) [77].
Xu et al. found that EAT volume acquired from contrast enhanced CT scans using a corrected threshold of –190 HU, –3 HU provided excellent agreement with EAT volume from non-contrast CT scans using a standard threshold of –190 HU, –30 HU [73].
The study by Xu et al. was interesting from the point of view of intensive care medicine, comparing EAT volumes acquired from 120 kVp ECG-gated cardiac non-contrast CT (NCCT) with non-ECG-gated chest NCCT and chest contrast-enhanced CT (CECT). The 120 kVp non-ECG-gated chest NCCT-1.25 mm images produced EAT volumes comparable to cardiac NCCT. Chest CT EAT volumes were excellent alternatives to the cardiac NCCT [80].
Given the necessity to perform CTCA with a thin slice thickness to allow assessment of the coronary arteries, manually contouring may be time-consuming [9]. Currently, there are emerging data that new artificial intelligence-based automated quantification of EAT is suitable for large-scale population studies. Commandeur et al. concluded that deep machine learning allows fast and fully automated quantification of EAT obtained from CT images for calcium scoring [79]. These authors stated that CT-acquired automated EAT quantification is ready for implementation in routine cardiovascular risk assessment. However, automated software to quantify EAT is not widely available.
Besides the assessment of EAT volume, there is growing interest in the CT radiodensity of EAT as a biomarker predicting adverse cardiac events [81]. A recent meta-analysis confirmed that patients with higher EAT radiodensity had a higher burden of coronary atherosclerotic disease [82]. A study demonstrated that EAT radiodensity was strongly associated with MACE [83].
Giabazzi et al. observed that increased EAT radiodensity was associated with MINOCA (myocardial infarction with nonobstructive coronary arteries and takotsubo syndrome) [84].
It has been suggested that increased EAT radiodensity of the pericoronary adipose tissue of unstable coronary plaques may reflect a higher metabolic activity of these coronary segments due to underlying inflammation. A PET-CT study demonstrated that the inflammatory activity of pericoronary adipose tissue (PVAT) was higher than in subcutaneous, visceral thoracic, or remote epicardial tissue in patients with non-ST segment elevation myocardial infarction (NSTEMI) [85].
Studies have shown good reproducibility of CCT measured EAT volume [86, 87]. Despite the well-validated association between EAT volume and CAD and atrial fibrillation (see Section 3), there is a lack of data regarding normal reference values of EAT volume [9]. Studies, searching for associations between CT-measured EAT volume and MACE, have often used the median value, tertiles, or quartiles of the EAT volume of the study population, rather than reference normal values for EAT volume to divide the study population for statistical outcome/survival analyses (see also Section 3) [71, 88–91].
A recent review pointed out that no meta-analysis has defined normal and pathological values for CCT-derived EAT volume and density [9]. Spearman et al. reported the threshold of CCT measured EAT volume > 125 mL as a predictor of cardiac pathology [92].
Echocardiography
EAT presents as the hyperechoic area between the ventricular wall and the parietal pericardium [93] (Figure 4). EAT is visually more prominent around the right ventricular free wall. EAT echocardiographic thickness is measured perpendicularly to the myocardial wall in the parasternal long axis, the short axis, and apical long-axis views [94]. A threshold value of 5 mm for EAT thickness has been proposed to identify individuals at higher cardiovascular risk [95].
FIGURE 4
Panel a shows a parasternal long axis view. Epicardial adipose tissue presents as hyperechogenic area between the pericardium (white arrow) and the myocardium. Panel B shows a parasternal short axis view. EAT is best detected at the right atrial free wall. The green line represents EAT thickness measurements. Red line indicates pericardial fat
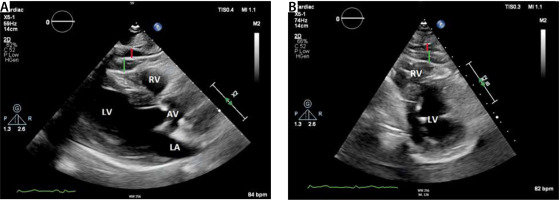
The advantages of echocardiography are its wide availability, relative cheapness, and no patient radiation exposure. The limitations of echocardiography assessed EAT are the inability of two-dimensional echocardiography to provide accurate volumetric EAT measurements and its high operator-dependency [96, 97]. Some authors have emphasised that EAT can be confused with pericardial effusion [97].
Cardiac magnetic resonance imaging
CMR allows volumetric EAT quantification with high reproducibility [10]. Different CMR imaging sequences can be used for EAT quantification (Figure 5). Contrary to CCT, CMR-acquired EAT estimation does not require patient radiation exposure. Assessment of EAT volume by CMR does not require gadolinium administration. For these reasons, CMR can be considered a safe technique to determine EAT volume if CMR contraindications are absent [98]. The drawbacks of CMR are the high cost, relatively long examination times, and limited availability. It should be emphasised that CMR has greater slice thicknesses than CCT, reducing EAT volume segmentation accuracy [9]. Furthermore, the depiction of the pericardium on the inferior CMR slices is more complex than on CCT axial images [9].
FIGURE 5
A 4-chamber view (Panel a) and 2-chamber view of the left ventricle from the cine views (SSFP sequence). Adipose tissue is bright in this imaging sequence. Note that it is more difficult to depict the pericardium on CMR compared to cardiac CT (see Figure 1). Note the EAT in the atrioventricular groove (green *) and pericardial outside the pericardium (red *)
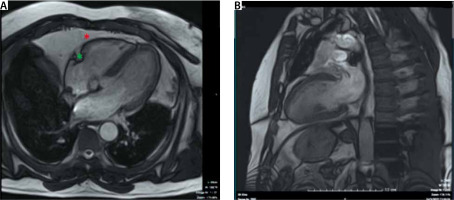
Table 1 summarises the advantages and disadvantages of CCT, CMR, and echocardiography for the assessment of EAT.
TABLE 1
Imaging features of echocardiography, cardiac CT and CMR impacting the EAT assessment
Section 3: EAT in clinical practice
EAT and coronary artery disease (CAD)
A meta-analysis, including 2872 patients, confirmed that EAT thickness and volume were significantly higher in patients with CAD than in patients without CAD (mean difference 1.57 mm, P < 0.00001; mean difference 15.22 mL, P < 0.0001, respectively). Compared to patients with lower EAT volume (< 100 mL), patients with higher EAT (≥ 100 mL) were more likely to have CAD, with a risk ratio of 0.69 (P = 0.01) [99].
Another meta-analysis, including more than 6600 patients from 7 studies, found that patients with myocardial infarction (MI) had 37% higher measures of EAT compared to patients without MI (confidence interval [0.21–0.54], P < 0.001). Furthermore, this analysis demonstrated the validity of the association between EAT thickness and MI irrespective of the imaging modality used. Similar relative differences in EAT with a wide overlap of the 95% confidence intervals were observed when studies using echocardiography and CT for EAT assessment were compared (for echocardiography: 0.4 [0.04–0.76], for CT: 0.36 [0.16–0.57], P < 0.001 for both) [100].
Monti et al. performed a meta-analysis (13 studies, 7683 subjects) that demonstrated that the mean EAT density was –86.40 HU (95% CI: –112.69 to –60.12 HU) in healthy subjects and –80.71 HU (95% CI: –87.43 to –73.99 HU) in patients with CAD [82].
In addition to the well-established relationship between EAT and the burden of CAD, several studies have sought to determine whether EAT volume can be used as a predictor of MACE, usually defined as a composite endpoint of cardiovascular death and non-fatal MI.
A prospective study included 245 patients who underwent a CTCA with EAT volume quantification. MACE was defined as myocardial infarction and cardiovascular death. During a 48-month follow-up, the hazard ratio of MACE was 1.4, 3.1, and 5.7 in the lower middle, upper middle, and highest quartiles of EAT as compared to the lowest quartile of EAT [89].
In an observational study of 843 consecutive HIV-infected patients, cardiovascular risk stratification was performed by cardiac CT-measured EAT volume and calcium score. During a mean follow-up of 2.8 years, 15 myocardial infarcts and 18 deaths occurred. The EAT volume and the coronary artery calcium (CAC score) by Agatston et al. [101] were higher in patients with events. The multivariable regression analyses demonstrated that the upper tertile of EAT and Agatston calcium score ≥ 100 were independent predictors of events after adjusting for age and sex [90].
Another meta-analysis, comprising 70 studies (41,534 patients), showed that subjects with any outcome of CAD had a higher mean EAT volume than those without CAD. Accordingly, it was de-monstrated that EAT volume was associated with obstructive stenosis, significant stenosis, any CAC, and MACE. EAT volume remained associated with obstructive stenosis (OR = 1.055), significant stenosis (OR = 1.514), myocardial ischaemia (OR = 1.062), and MACE (HR = 1.040) in multivariable models [102]. Furthermore, EAT volume was found to be associated with CTCA-assessed high-risk plaque features. A meta-analysis, including nine studies (3772 patients), demonstrated that increasing EAT was significantly associated with the presence of high-risk plaques (HRP) with OR of 1.26 (95% CI: 1.11–1.43; P < 0.001). Compared to patients without HRP, patients with HRP had higher EAT volume (P < 0.001) [103].
A recent meta-analysis (29 articles; 19,709 patients) found that increased EAT thickness and volume were associated with higher risks of cardiac death (OR = 2.53; P = 0.020), myocardial infarction (OR = 2.63; P = 0.003), coronary revascularization (OR = 2.99; P < 0.001), and atrial fibrillation (adjusted OR = 4.04; P < 0.001) [104].
A study with a median follow-up of 40.4 months included 117 patients for whom CTCA and invasive coronary angiography data were available [105]. Statistically significant differences in EAT volume, CTCA ≥ 50% stenosis, and a positive CT-FFR were found between patients developing MACE and without MACE (all P < 0.05). The adjusted multivariable analysis demonstrated that EAT volume and indexed EAT volume (HR = 2.21, P = 0.023; HR = 2.03, P = 0.035, respectively) were predictors of MACE. Furthermore, this study showed that the addition of CTCA ≥ 50% stenosis and EAT to clinical risk assessment by the Morise score [106] improved the C-index compared to the Morise score alone (AUC 0.83 vs. 0.66, P = 0.004) [105].
EAT and AF
Over the last decade, the associations between EAT volume, EAT density (CT attenuation coefficient), and AF or its recurrence have been extensively studied. Table 2 summarises the studies searching for associations between EAT volume, EAT density, and AF, published between January 2020 and March 2022 [107–125]. Most of these latter studies found that a higher EAT volume, both total-EAT volume and LA-EAT (EAT surrounding the left atrium) volume, is associated with a higher prevalence of AF, a higher incidence of postoperative atrial fibrillation (POAF) after coronary artery bypass graft (CABG), and a higher AF recurrence rate after AF ablation. A higher EAT density was associated with AF and AF recurrence. It has been postulated that higher CT attenuation values of EAT may reflect inflammatory active fat tissue.
TABLE 2
Human studies searching for associations between EAT volume and EAT density and atrial fibrillation, published between January 2020 and March 1, 2022
Author, year and reference | Number of patients and type of study | Results |
---|---|---|
Slipczuk et al., 2021 [107] | Retrospective, post hoc analysis of 379 patients with a confirmed COVID-19 diagnosis | 4.22% of the patients developed new-onset AF (4.22%). Patients who developed AF during the index admission had higher EAT (P = 0.049). Patients with new-onset AF had worse clinical outcomes (death/intubation/vasopressors) (87.5 vs. 44.1%; P = 0.001). |
Yang et al., 2022 [108] | 506 patients with AF recurrence and 174 patients without AF recurrence. Retrospective study | Total-EAT density (HU) value (P < 0.001) and LA-EAT density (HU) value (P < 0.001) were significantly higher in the patients with AF recurrence than in those without recurrence. In a multiple logistic regression analysis, a higher LA-EAT density (OR = 1.12; 95% CI: 1.02-1.22, P = 0.015) was significantly associated with the AF recurrence after adjusting for other risk factors. |
Rao et al., 2021 [109] | 77 consecutive adults with end-stage HF prior to LVAD. Retrospective study | Study groups were divided into above-median (≥ 219 cm3) and below-median (< 219 cm3) pericardial adipocyte tissue (PAT) volume. Patients with above-median PAT had a higher proportion of atrial fibrillation, chronic kidney disease and ischaemic cardiomyopathy. PAT is not associated with worse 2-year LVAD-related outcomes. |
Gaibazzi et al., 2021 [110] | 80 patients who underwent AF ablation and 80 patients without history of AF | Mean fat density was significantly higher in AF versus non-AF (P < 0.0001) participants. In the logistic regression models, only the addition of mean fat-LA attenuation led to a significant improvement of the model’s χ2 (from 22.89 of the clinical model to 31.69 of the clinical and adipose tissue attenuation model, P < 0.01) and discrimination (AUC from 0.775 to 0.829). |
Beyer et al., 2021 [111] | 732 patients with nonvalvular AF who underwent cardiac CTA before PVI | Epicardial adipose tissue volume in patients with AF recurrence was higher (P < 0.0001) and further progressed significantly in a subset of 85 patients after 2 years (P = 0.041). Attenuation levels were lower, indicating a higher lipid component associated with AF recurrence (P = 0.001). |
Eren et al., 2021 [112] | 413 consecutive patients diagnosed with STEMI | Echocardiography measured EAT thickness was higher in the group with atrial fibrillation than in the control group (P < 0.001). The SYNTAX risk score was higher in the atrial fibrillation group (P < 0.001). In the logistic regression analysis, EAT was detected to be an independent predictor for the development of atrial fibrillation (OR = 4.135, P < 0.001). |
Eren et al., 2021 [113] | 493 consecutive patients who were diagnosed with NSTEMI | Echocardiography measured EAT thickness was higher in the AF group than in the controls (P < 0.001). The TIMI risk scores were higher in the AF group (P < 0.001). Logistic regression analysis showed that EAT was an independent determinant for the development of AF (OR 3.521, P < 0.001). |
Hammache et al., 2021 [114] | 389 patients undergoing first-time RF PAF ablation | Neither tot-EAT volume (P = 0.519), tot-EAT radiodensity (P = 0.892) nor La-EAT radiodensity (P = 0.556) was significantly associated with AF recurrence after PVI. |
Ischii et al., 2021 [115] | Left atrial appendages (LAA) were obtained from 76 consecutive AF patients during cardiac surgery | CT images demonstrated that the %change in EAT fat attenuation was positively correlated with EAT fibrosis, determined on LAA histology. |
El Mahdiui et al., 2021 [116] | 460 consecutive patients with symptomatic AF referred for first AF catheter ablation who underwent computed tomography were included | Patients with higher attenuation (≥ –96.4 HU) of the posterior LA adipose tissue showed higher AF recurrence rates compared with patients with lower attenuation (< −96.4 HU; log-rank test P = 0.046). On multivariable analysis, posterior LA adipose tissue attenuation (HR = 1.26 [95% CI: 0.90–1.76]; P = 0.181) remained a promising predictor of AF recurrence following catheter ablation. |
Zhou et al., 2021 [117] | 93 consecutive patients with hypertrophic obstructive cardiomyopathy (HOCM) | In multivariable logistic regression analysis, EATVI, LAVI, and LVEF remained independent determinants of AF occurrence (OR = 1.023, P = 0.023, OR = 1.043, P = 0.006, and OR = 0.887, P = 0.004, respectively). |
Zhu et al., 2021 [118] | 2042 patients referred for coronary computed tomography angiography (CCTA) | AF was present in 8.5%. The patients with non-valvular AF had significantly higher BMI and EATV with more pronounced elevation of EATV (P < 0.001). Multivariate logistic regression showed a significant association of EATV with AF after adjustment for BMI and clinical risk factors, and the highest EATV quartile was associated with AF independently of left atrial size and obstructive coronary artery disease. |
Guzel et al., 2020 [119] | A total of 79 patients who underwent haemodialysis (HD) for periods longer than 3 months were included | The mean EFT thicknesses were measured as 7.2 ± 2.3 mm. A positive correlation was found between the EAT thickness and age, C-reactive protein, and left ventricle rear wall thickness. AF was found in 18 (22.8%) patients in the Holter ECG examination. When the group with AF was compared with the non-AF group, there were no significant differences in terms of epicardial adipose tissue thickness and other parameters. |
Nakatani et al., 2020 [120] | 44 nonparoxysmal AF patients underwent left atrial posterior wall isolation (LAPWI) after pulmonary vein isolation | No differences were found between the AF-free and AF-recurrent groups regarding EAT volume. Although no difference was found between groups regarding the EAT overlap on LAPWI area, the AF-free group had a significantly larger EAT overlap on LAPWI lines (1.4 ± 1.0 vs. 0.6 ± 0.6 cm2, P = 0.014). Multivariate analysis identified EAT overlap on LAPWI lines as an independent predictor of AF recurrence (HR = 0.399, P = 0.025). |
Cosansu et al., 2020 [121] | Cross-sectional study (80 AF patients with acute ischaemic stroke [AIS] and 80 age-gender matched AF controls) | In comparison with the control group, AF patients with AIS had significantly higher EAT thickness (P < 0.0001). The multivariate regression analysis indicated that EAT independently predicted AIS in patients with AF. |
Gunturk et al., 2020 [122] | 124 patients scheduled for on-pump CABG | In multivariate logistic regression analysis, echocardiography measured EAT was found to be an independent predictor for development of POAF (OR = 4.47, 95% CI: 3.07–5.87, P = 0.001). |
Kawasaki et al., 2020 [123] | 64 paroxysmal AF patients without HF undergoing catheter ablation (CA) | The volumes of total EAT and periatrial EAT surrounding the left atrium were measured by computed tomography before CA, and the periatrial to total EAT volume ratio (P/T) was obtained. During the follow-up period of 11 ± 4 months, AFR was observed in 14 patients. The P/T was significantly greater in patients with than without AFR. Greater P/T was determined by ROC curve analysis to be independently associated with AFR. |
Yamaguchi et al., 2020 [124] | Single-centre retrospective study enrolling 202 patients who underwent both TTE and TEE | EAT thickness was measured on TTE in parasternal long-axis view. LAA orifice areas were measured in 41 patients with 3-dimensional TEE data. There was a significant negative correlation between EAT thickness and LAA emptying flow velocity (ρ = –0.56, P < 0.001) and a significant positive correlation between EAT thickness and LAA orifice area (ρ = 0.38, P = 0.014, respectively). The best EAT thickness cut-off value for low LAA emptying flow velocity was > 5.1 mm (c-statistics, 75.7%). A thickened EAT was associated with low LAA emptying flow velocity, which increases the risk of thromboembolic phenomena in the presence of AF. |
Liu et al., 2020 [125] | 37 persistent AF patients who underwent CABG and a group (n = 37) matched by age, gender, and body mass receiving CABG | The EAT thickness in patients with persistent AF was significantly greater than that in the control group (P = 0.02). There were no significant differences in plasma levels of IL-1β and adiponectin between the 2 groups. Multiple logistic regression analysis showed that IL-1β was an independent risk factor of persistent AF. |
A meta-analysis, including ten case-control studies, found that EAT volume, both total-EAT volume and EAT volume surrounding the left atrium (LA-EAT), was significantly increased in AF patients. There was a statistically significant difference in the total-EAT volume subgroup and in the LA-EAT volume subgroup between patients with persistent AF (PeAF) and paroxysmal AF (PAF) [126].
Another meta-analysis collected data from 10 studies (546 patients) searching for an association between EAT and postoperative AF (POAF) after cardiac surgery. It was found that EAT was higher in those with POAF irrespective of the type of EAT measurement (total EAT volume, EAT thickness) [127].
Shamloo et al. reported the results of a meta-analysis that included data from 12 studies that searched for an association between EAT volume and recurrence of AF after AF ablation therapy. The results showed that LA-EAT and total EAT volumes were higher in patients with AF recurrence. Furthermore, higher EAT thickness was associated with AF recurrence [128].
EAT and valvular heart disease
A study including 225 patients admitted for coronary angiography due to new-onset angina measured the EATTTE by transthoracic echocardiography. Patients with an EATTTE ≥ 7 mm were found to be older and more often had hypertension and hyperlipidaemia. The average aortic valve sclerosis score index was higher (P = 0.001), and LV ejection fraction (EF) and early mitral annular velocity were significantly lower in patients with EATTTE ≥ 7 mm. LV hypertrophy was more prevalent in patients with EATTTE ≥ 7 mm (P = 0.026). This study concluded that EAT might promote inflammatory and atherosclerotic modifications in the aortic valve [129].
In a study cohort of 200 patients with severe aortic stenosis (AS) and 200 control patients, Mahabadi et al. observed that EATTTE was significantly and independently associated with severe AS [130]. The logistic regression analysis showed that an increase in EAT by one standard deviation was associated with a two-fold increased prevalence of AS. After adjustment for gender, age, and traditional cardiovascular risk factors, this association between EATTTE and severe AS remained confirmed [130]. Again, it was concluded that these findings support the notion that EAT may promote sclerosis of the aortic valve.
A study searching for a relation between valvular calcium deposits and EATTTE included 294 patients who underwent non-contrast chest CT. EATTTE was measured at various locations. Mitral annular calcification (MAC) and aortic valve calcification (AVC) were quantified using the Agatston technique. After adjusting for clinical variables, including body mass index (BMI), EATTTE at the superior interventricular groove remained significantly associated with total valvular calcium [131].
Studies exploring possible associations between EAT volume and clinical outcomes in valvular disease are relatively rare. Given the availability of chest CT in transcatheter aortic valve implantation (TAVI) patients as part of the workup prior to TAVI, most studies searching for an association between EAT volume and outcome have been performed in TAVI patients.
In patients undergoing TAVI, Weferling et al. found that a larger EAT volume was independently associated with pre-existing first-degree AV block and new pacemaker implantation after TAVI (OR = 1.005; P = 0.035) [132]. Another study with a small sample size did not detect an association between EAT volume and the need for pacemaker implantation after TAVI [133].
Associations between EAT and heart failure and LV remodelling
The effects of EAT on left ventricle mass (LVM) have been studied in echocardiography, CMR studies, and autopsy series [13, 134]. These series have systematically reported an association of EAT with LVM in normal subjects and patients with CAD and hypertrophic cardiomyopathy (CMP) [13].
Interestingly, studies focusing on LV remodelling in ischaemic and non-ischaemic dilated CMP demonstrated that in patients with dilated CMP, the EAT-to-LVM ratio was reduced compared to healthy subjects [135, 136]. Nonetheless, a positive correlation between LVM and EAT was found even in the patients with dilated CMP [135]. Although no significant correlation between LV EF and EAT was found in patients with dilated CMP, EAT was associated with a more beneficial LV remodelling process, expressed by a less disturbed LV concentricity index (LVM/LVEDV; LVEDV = LV end-diastolic volume) [135].
There are conflicting data on the association between EAT and LV function. In contrast to the abovementioned study, Khawaja et al. found that a stepwise decrease in LV EF was associated with decreasing EAT volume [137].
Although the contribution of EAT to the pathogenesis of LV remodelling is not fully understood, studies have suggested a potential role of EAT in heart failure, especially in heart failure with preserved LV EF (HFpEF) [135, 138–140]. In some studies the EAT volume was found to be higher in patients with HFpEF after ruling out the potential confounding effects of obesity and CAD [138, 139]. Iacobellis emphasised that the association between EAT volume and heart failure with reduced LV EF (HFrEF) remains controversial due to the inconsistency between the reported findings among the different studies [141].
A recent systematic review exploring the significance of EAT thickness (EATth) measurements in correlation with B-type natriuretic peptide levels (BNP/NT-pro-BNP) as diagnostic and prognostic markers in patients with or at risk of heart failure included 12 studies (1983 individuals). There was a strong association between increased EATth and increased BNP/NT-proBNP levels in patients with metabolic disease. The reviewers concluded that EATth is a valuable tool for diagnosing and determining the prognosis of heart failure [142].
Studies on EAT and prognostic outcomes in specific clinical settings: patients hospitalised in the ICU or after elective surgery
The medical literature on the utility of EAT volume to predict clinical outcomes in patients hospitalised in the ICU or undergoing major elective surgery is somewhat limited.
A study searching for a correlation between EAT volume and outcome 30 days after elective non-cardiac surgery found that patients in the third BMI adjusted EAT (EAT/BMI) tertile had an HR of 2.8 for the composite endpoint of non-fatal myocardial infarction and cardiovascular death, compared to patients in the first EAT/BMI tertile [91].
Another study demonstrated that EAT thickness (EATth) predicted in-ICU complications after CABG. Most in-ICU patients suffered from various events, which were mainly insignificant. Nevertheless, 29.4% of the events were scored as clinically relevant. The most common complication was AF, with a reported incidence of 9%. A statistically significant difference in mean EATth was found between patients with and without in-ICU complications, with EATth of 7.64 and 6.16 mm, respectively (P = 0.015). It was found that the best threshold of EATth for predicting in-ICU complications was 6.5 mm, with a sensitivity of 65.9% and specificity of 58.8% [143].
Another study that searched for clinical factors promoting POAF after cardiac surgery found that loop diuretics had the most robust association with POAF. The logistic regression analysis demonstrated that a high left atrial EAT/Total EAT ratio was the second strongest predictor of POAF [144].
A study including patients with confirmed COVID-19 pneumonia on CT sought a correlation between prognosis and EAT volume or EAT attenuation (EAT-At). The latter was a marker of EAT inflammation. The primary outcome was a composite endpoint: referral to the ICU, invasive ventilation, or death. A multivariable analysis, including diabetes mellitus, overweight/obesity, arterial hypertension, and EAT characteristics, revealed EAT-At to be an independent predictor of critical illness. Increased EAT-At was found to be a predictor of unfavourable outcomes in patients with critical COVID-19 infection. However, neither obesity nor EAT volume predicted critical COVID-19 infection [145].
Another study concluded that EAT volume assessment on chest CT at hospital admission in patients with COVID-19 might be helpful for risk stratification of disease aggravation. The authors demonstrated that ICU admission or death (14%) was associated with EAT volume (P = 0.015), hypertension, and ≥ 25% lung involvement on chest CT. The association between EAT volume and severe COVID-19 remained statistically significant after adjustment for sex, BMI, lung involvement, and ferritin levels but not after age adjustment [146].
Millman et al. investigated the contribution of cardiac and lung ultrasound and clinical variables by developing a straightforward point-of-care POCOVID score system to predict short-term mortality in critical COVID-19 patients [147]. They showed that deceased patients were generally older, had worse values for SOFA score, increased baseline troponin levels, reduced left ventricular ejection fraction (LVEF), impaired LV diastolic function, and increased EATth, despite a similar prevalence of severe lung ultrasound scores. The POCOVID score, including age (> 60 years), myocardial injury (LVEF < 50% or TnI > 99th percentile), and increased EATth (> 0.8 cm), was created. The presence of two out of these three criteria identified patients with almost twice the risk of death [147].
CONCLUSIONS
EAT volume and EAT radiodensity are new CCT-acquired biomarkers. The association between EAT volume and CAD has robustly been validated. EAT volume and EAT radiodensity are predictors of myocardial infarction. Meta-analyses, including large numbers of patients, provide strong evidence that increased EAT volume is associated with a higher risk of myocardial infarction, the need for coronary revascularization, and cardiovascular mortality.
EAT volume predicts the occurrence and reoccurrence of atrial fibrillation after cardiac surgery and pulmonary vein isolation. Meta-analyses robustly validate the association between increasing EAT volume and the occurrence/reoccurrence of AF.
There is substantial evidence for an association between EAT volume and adverse clinical outcomes in patients with HFpEF.
Studies have demonstrated that increased EAT volume in patients with COVID-19 infection, requiring treatment in the ICU, is associated with poor outcomes.
There is growing evidence that EAT is associated with aortic valve stenosis/calcification and mitral annular calcification, but further research is needed to determine the exact role of EAT in the progression of valvular heart disease.
Overall, the association between EAT volume and MACE has been robustly validated over the last decade. Reliable semi-automated and automated software quantifying CT-acquired EAT volume has been developed. Therefore, CCT-assessed EAT volume seems a promising biomarker to predict MACE.