Purpose
High-dose-rate (HDR) gynecological brachytherapy provides a conformal local dose, which is effective for treatment of cervical cancer, and is commonly combined with external beam radiation therapy (EBRT) [1]. Across multiple institutions, EBRT combined with magnetic resonance imaging (MRI) or computed tomography (CT)-based brachytherapy has been extremely effective in the treatment of locally advanced cervical cancer treatment [2]. The large dose gradient, which HDR brachytherapy sources offer, is accompanied by an inherent sensitivity to source positioning errors, with significant deviations in planned dose to organs at risk (OARs) and target volumes, associated with a few millimeters of error [3, 4]. Modern gynecological brachytherapy utilizes three-dimensional (3D) imaging techniques that enable superior delineation to enhance local control and limit toxicity to OARs [5]. The multi-stage 3D image-guided gynecological brachytherapy workflow presents an array of uncertainties and potential errors, such as implant displacement, reconstruction and image fusion uncertainties, and catheter swap errors [6].
Image-guided brachytherapy for cervical cancer necessitates complexities that require thorough treatment verification. In vivo dosimetry methods will enable an evaluation of the applied dose distribution. Fonseca et al. concluded within a review of the requirements and future developments for in vivo dosimetry in brachytherapy that dosimetry, which can provide live dose readout, has an increased likelihood of resolving deviations from the treatment plan. Additionally, a live readout system will enable interruption or adaption of treatments in response to an observed error [7]. HDR source tracking provides dwell position and timing information for treatment verification. Real-time source tracking is pursued within the brachytherapy community through multiple methods. A point detector-based approach, where individual dosimeters are used to triangulate source position, has been established [8, 9]. The recent progression of diode arrays and flat panel systems through HDR brachytherapy phantom validation, suggests imminent clinical implementation once clinical trials have commenced [10-13].
Clinical application of real-time in vivo source tracking systems requires a specific error threshold. Treatment termination or real-time adjustment is essential during treatment verification and is accomplished through the definition of an in vivo source tracking error threshold. Poder et al., established the ‘in vivo source tracking error threshold’ for trans-rectal ultrasound (TRUS)-based HDR prostate brachytherapy, by determining a deviation of source position from the treatment plan, which causes treatment failure [14]. The study provided recommendations for TRUS-based prostate brachytherapy to enable the implementation of source tracking technology, and provide insight into the complexity of defining a single in vivo source tracking error threshold, given variation in patient anatomy and plan optimization. Distinct differences between prostate treatment and image-based gynecological brachytherapy exist in the applied dose distribution, anatomy, treatment workflow, and treatment endpoints, which necessitate additional investigation.
Previous studies evaluating the effect of dwell position displacement in HDR gynecological brachytherapy have focused particularly on displacements along the superior-inferior direction [4], or along the applicator channel [3], with the purpose of demonstrating the impact of reconstruction uncertainties. In order to establish a complete in-vivo source tracking error threshold for cervical brachytherapy, an evaluation of intra-cavitary and interstitial treatments in all directions is necessary. 3D image-guided brachytherapy for cervical cancer presents possible uncertainty in all directions, due to co-registration error, applicator displacement during patient transport, and imaging artefacts [15, 16]. Currently, no study exists assessing both interstitial and intra-cavitary treatments for dwell position displacement in each direction. To define an acceptable threshold of error during treatment and the impact dwell position error has on treatment endpoints, needs to be evaluated along each axis.
The purpose of this study was to determine a comprehensive in vivo source tracking error threshold and to validate its’ application in HDR brachytherapy for cervical cancer. A dwell position displacement, which causes a detrimental increase in dose to the bladder and rectum and a critical reduction in dose to high-risk clinical treatment volume (HR-CTV), was determined for interstitial and intra-cavitary treatments. Achieving this enables the definition of an action level along each axis, for which the treatment is interrupted, preventing clinically relevant changes to the applied dose.
Material and methods
Treatment protocol
Three-dimensional (3D) image-based HDR gynecological brachytherapy, performed at the Prince of Wales Hospital, Nelune Comprehensive Cancer Centre (NCCC), involves surgical insertion of the applicator and prescribed additional needles for interstitial treatments. Fletcher CT-MR applicator set 189.730 (Elekta Brachytherapy, Veenendaal, The Netherlands) and lunar ovoid Venezia advanced gynecological applicator 3905TH (Elekta Brachytherapy, Veenendaal, The Netherlands) were used for intra-cavitary and interstitial cases within this study. A 3D image of a patient was acquired with organ, high-risk, and intermediate-risk volumes delineated via MRI (1.5 T Siemens, T2 spin echo). Applicator libraries were utilized to reconstruct channel position during treatment planning. Cases, which required needles to enable adequate CTV coverage also underwent CT imaging with 2 mm slice thickness. A marker wire was placed within the interstitial needles to assist CT visualization and localize the correct source positions along the needle. High-risk clinical treatment volume (HR-CTV), intermediate-risk clinical treatment volume (IR-CTV), and bladder and rectum volumes were contoured on transverse slices according to GEC-ESTRO protocol [17]. CT dataset was rigidly fused based on applicator position with a T2-weighted MRI sequence, which contained the contours. Once the applicator model and/or interstitial needles were reconstructed within Oncentra Brachytherapy treatment planning system (v. 4.6.0 Elekta, Stockholm, Sweden), graphical optimization (GrO) of dwell weightings were carried out. Cumulative dose to critical volumes were considered given the accompanied EBRT fractions, with total dose constraints for the critical volumes using a spreadsheet. Patients, who were treated prior to implementing the EMBRACE II protocol were prescribed dose based on a total dose limit to the bladder and rectum, which implemented a 10% probability of G2-G4 side effects [18, 19].
Patient treatment data
Thirty patients, who were previously treated at the NCCC for cervical cancer were randomly selected to determine the feasibility of implementing an in vivo source tracking error thresholds, which can protect from dwell position uncertainty. Patients of 18 years of age or over were selected for the study, who have undergone brachytherapy for cervical cancer stages IIB, IIIA, or IIIB. Ten interstitial and twenty intra-cavitary treatments were assessed. Interstitial patients were treated with Fletcher or Venezia applicators, equipped with the required needles. On average, 5 (ϭ = 2.2) needles were used, with plans ranging from 2 to 9 needles. Intra-cavitary cases included only the tandem-ovoid Fletcher applicator. Patients have had brachytherapy as a local boost to accompany their external beam therapy and chemotherapy treatments. A single fraction of a 3 to 4 fraction treatment was considered for each case. The required Southeastern Sydney Local Health District Ethics approval has been granted, under the Department of Radiation Oncology research authorization number: SESLHD-NRA REF: 2021/16.
Digital imaging and communications in medicine (DICOM) plan and structure set files were exported from Oncentra Brachytherapy treatment planning system for each patient. Treatment plan information as well as the bladder, rectum, and HR-CTV contours were extracted from patients’ DICOM files. An example of the exported structure set and treatment plan is presented in Figure 1. The shape of treatment plan isodose volume and its’ proximity to OARs would determine the in vivo source tracking error threshold.
Fig. 1
A) Interstitial treatment with needles and three applicator channels shown in magenta; B) Intra-cavitary treatment with three applicator channels. For interstitial (A) and intra-cavitary (B) treatment plans, the 100% isodose volume is shown in blue. Contours from patient dataset are displayed with HR-CTV volume in red, bladder in yellow, and rectum in brown
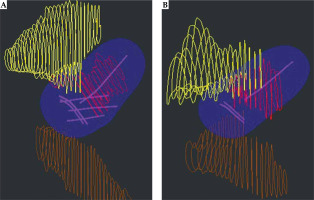
Simulation of localization uncertainty
To simulate the displacement of dwell positions, 3D coordinates of each planned dwell position were shifted within DICOM treatment plan. Dose to the bladder, rectum, and HR-CTV were calculated without re-optimization. A displacement of dwell positions and re-calculation of applied dose without re-optimization allowed for simulation of source positioning errors. To displace dwell positions, a Python script was developed using pydicom package version 2.2.1. (Darcy Mason, pydicom contributors).
The script alters the 3D position of all dwell positions in treatment planning system (TPS) coordinate system. All dwell positions were displaced in unison along anterior-posterior (Ant-Post), superior-inferior (Sup-Inf), or right-left directions individually. Displacements of ±7 mm were implemented in 1 mm increments along each axis for all dwell positions in the plan, with a new treatment plan created every increment, producing a total of 1,260 new plans. Within the image-based gynecological brachytherapy workflow, dwell position errors can occur in any direction. Examples of these uncertainties include source positioning accuracy, applicator displacement, needle reconstruction error, needle swap errors, uncertainty in image fusion, and an incorrect indexer length entered into TPS.
Applicator rotation for intra-cavitary cases was also considered to quantify the impact of failure following surgical insertion. Inadequate or imbalanced surgical gauze packing around the applicator can cause applicator rotation during patient transport, or when moving the patient on and off imaging equipment. A change in applicator position cannot be accounted for once imaging has taken place. A rotation of the applicator about the intra-uterine tube (tandem) was considered for intra-cavitary cases (n = 20), as shown in Figure 2. Interstitial cases (n = 10) were not considered for rotational errors, as it was assumed that needles, which pass through the ovoids and into the tissue should minimize this risk of applicator rotation. The tandem was defined as an axis of rotation for the applicator. A Python script was developed to simulate applicator rotation by rotating all dwell positions about the tandem, ±20° in 5° increments. Current literature considers a shift in the dwell positions along a ring applicator channel when evaluating channel reconstruction uncertainties, and not the rotation of a tandem-ovoid applicator set [4].
To calculate the change in OARs dose and HR-CTV coverage for each patient, the original treatment plan, structure set, and altered treatment plans were imported into RadCalc, a secondary check program (version 3.6 Lifeline Software, Austin, USA). RadCalc is clinically used as an independent QA of treatment plans to verify the dose delivered. The program utilizes TG-43 formalism to determine the dose to critical volumes and dose points. A dose calculation space with a 10 mm expansion margin around each structure was defined, with a 1 mm3 voxel size implemented for discretization. Treatment plan parameters calculated in RadCalc were all within 0.2% of those determined in Oncentra Brachytherapy treatment planning system for the clinical dataset used within this study. Individually, each treatment plan was opened and the dose to the bladder, rectum, and HR-CTV volumes were calculated.
DVH parameters utilized within GEC-ESTRO recommendations for treatment endpoints were calculated to evaluate the change in dose to clinical volumes [5]. D2cc indicated the dose received by the most irradiated two cubic centimeters of each OAR. D90 parameter was used to determine HR-CTV coverage and signified the dose to 90% of the volume. Parameters were calculated for a single-fraction; the delivery of subsequent fractions was not considered when calculating the in vivo source tracking error threshold.
Results
Original treatment plans
Box plots were used throughout to demonstrate the distribution of the patient population. The box plot has an interquartile range, which spans 50% of the patient population. In Figure 3 and throughout, the red line signifies the median, with the lower and upper edges of the box illustrating the 25th (Q1) and 75th (Q3) percentile, respectively. The maximum and minimum lines span up to 1.5 times the interquartile range, with results outside this range taken to be outliers, and illustrated with a red ‘+’ symbol.
Fig. 3
Dose given to bladder (A), rectum (B), and HR-CTV (C) by un-altered treatment plans. Box plots represent the distribution of doses provided by the un-altered treatment plans
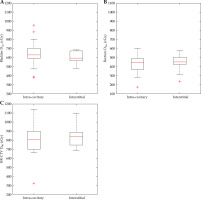
Figure 3 demonstrates the dose parameters of the original unmodified treatment plans. Generally, the bladder was allowed a higher dose than the rectum by the treatment protocols used, which was consistent within the dataset in both interstitial and intra-cavitary cases.
A relative increase in D2cc for an OAR can be detrimental to patient health; similarly, a 10% reduction in the D90 for HR-CTV can diminish local control. Therefore, the action level of a 10% relative change to more than 25% of the patient dataset was established. Graphically, when the marked 10% threshold was within the box of the box plot, a minimum of 25% of the patients would receive more than a 10% relative change in the dose for that displacement. A set in vivo source tracking limit required an evaluation of HR-CTV coverage and the dose to OARs based on a single fraction of the multi-fraction treatment plan. EMBRACE II recommends combined dose constraints for EBRT and multiple brachytherapy fractions [18]. Consequently, determining a real-time displacement threshold based upon absolute dose was inappropriate, since only a single fraction can be assessed per plan, and thus dwell position displacement was evaluated with a relative measure for this study. In line with the literature, a 10% change in prescribed dose to OARs or HR-CTV was applied as a critical threshold [3].
Intra-cavitary treatments
A positive displacement translated to a posterior shift when considering the anterior-posterior axes, superior shift for the superior-inferior axes, and displaced the dwell positions left for the right-left axes, with a negative displacement corresponding to the opposite directions.
A relative change in D2cc for the bladder due to displacement in the Ant-Post direction is demonstrated in Figure 4A, and reveals a 2 mm displacement that meets the threshold. A shift in dwell position was most sensitive along this axis. A minimal increase in dose to the bladder was seen for shifts in all other directions, with the error threshold only met with a 6 mm shift in the inferior direction. There were multiple outliers, which were particularly sensitive to displacement in the right-left and superior-inferior direction.
Fig. 4
Relative change in bladder D2cc, with displacement in the anterior-posterior (A), right-left (B), and superior-inferior (C) directions for intra-cavitary cases. Red dashed line denotes the threshold, a 10% relative increase in dose
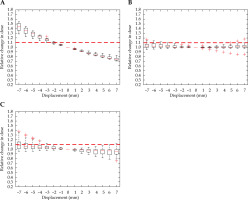
Similar to Figure 4, Figure 5 indicates that the rectal D2cc was most sensitive in the Ant-Post direction, reaching a 10% increase in the dose with a 2 mm shift. Dwell position displacement along the other two axes failed to cause a significant increase in the dose to more than 25% of the cohort.
Fig. 5
Relative change in rectum D2cc, with displacement in the anterior-posterior (A), right-left (B), and superiorinferior (C) directions for intra-cavitary cases. Red dashed line denotes the threshold, a 10% relative increase in dose
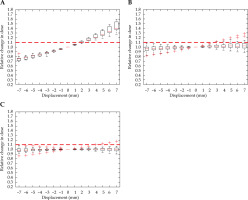
Figure 6 demonstrates a shift in dwell position that has a measurable effect on the HR-CTV D90 parameter in every direction. There was a little directional dependence in the transverse plane for HR-CTV coverage. A shift of 4 mm in the anterior-posterior direction as well as a 5 mm shift to the left and right, caused a significant detriment to local control. Displacement of the dose distribution in the inferior direction stimulated a significant decrease in coverage at 5 mm. An increase in D90 occurred with superior displacement, and was the result of ovoid channels entering the HR-CTV. In some cases, the applicator movement superiorly would be bound by the external os of the cervix.
Fig. 6
Relative change in HR-CTV D90, with displacement in the anterior-posterior (A), right-left (B), and superior-inferior (C) directions for intra-cavitary cases. Red dashed line denotes the threshold, a 10% relative decrease in dose
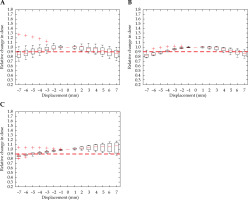
Outliers generally decreased from multiple patients without a relationship across different volumes or directions of displacement. An exception being the outliers presented in the Ant-Post and Sup-Inf directions for the HR-CTV (Figure 6A, C). Additionally, the same plan elicited an outlier for the rectum, as presented in Figure 5C. Outliers were not dissimilar with respect to initial HR-CTV coverage or OAR dose to the rest of the dataset.
Figure 7 demonstrates applicator rotation having a minimal dose difference to all volumes considered. The bladder reached its’ dose threshold at –20°, and the rectum overcomes the set tolerance at 20°. Due to the rotational symmetry in the para-axial plane of the HR-CTV contour and pear-shaped dose distribution, applicator rotation presented a minimal effect on HR-CTV coverage within the ±20° tested. The contour and dose distribution are illustrated in Figure 1A.
Interstitial treatments
The majority of interstitial cases produced results similar to intra-cavitary treatments, but were more sensitive in some instances, as dwell positions could exist closer to OARs. Figures 8 and 9 show interstitial plans, which are identical to intra-cavitary plans for the rectal and bladder critical limits of 2 mm and –2 mm, respectively, when considering the anterior-posterior direction. The interstitial plans were more sensitive to displacement in the superior-inferior directions for both OARs, with the bladder displacement limit of a 4 mm inferior shift and with rectal limit of a 5 mm superior shift.
Fig. 8
Relative change in bladder D2cc, with displacement in the anterior-posterior (A), right-left (B), and superior-inferior (C) directions for interstitial cases. Red dashed line denotes the threshold, a 10% relative increase in dose
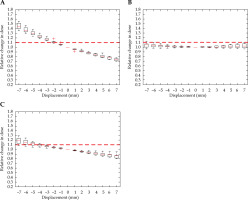
Fig. 9
Relative change in rectum D2cc, with displacement in the anterior-posterior (A), right-left (B), and superior-inferior (C) directions for interstitial cases. Red dashed line denotes the threshold, a 10% relative increase in dose
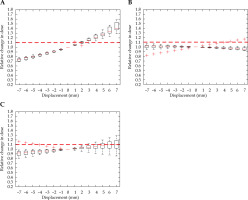
Figure 10 demonstrates a critical displacement for the HR-CTV in each direction. A respective –5 mm and 4 mm shift in the anterior-posterior direction, a –4 mm and 5 mm displacement in the right-left direction, and a –5 mm and 6 mm shift in the superior-inferior directions. The individual sensitivity to displacement of each case was demonstrated by the outliers in the superior-inferior direction, where two cases experienced a detrimental reduction in the HR-CTV coverage.
Discussion
A comprehensive evaluation of the in vivo source tracking thresholds for image-guided HDR gynecological brachytherapy provided insight into the impact of dwell position displacement on treatment endpoints. The in vivo source tracking error thresholds is directionally dependent and can vary greatly from patient-to-patient.
Variation in initial doses within the dataset can be explained by the differing needs of each patient, considering the extent of disease, differences in anatomy, and the dose history of the patient. Most notably, all cases examined are treatment plans from a single fraction, which is part of a three to four fraction treatment schedule, where dose escalation or de-escalation can occur during the following fractions. Within the governing recommendations, dose constraints are set for the whole treatment schedule, combining all EBRT and brachytherapy fractions [18]. Consequently, determining a threshold displacement based upon absolute dose was inappropriate, since only a single fraction could be assessed per plan, and thus dwell position displacement was evaluated with a relative measure for this study [4]. In line with the literature, a 10% change in prescribed dose to organs at risk or the HR-CTV was prescribed as a critical threshold [3].
Outliers for intra-cavitary cases were most likely due to anatomical differences between the patients, since a similar three-channel configuration existed for the tandem and ovoid-based applicators across all the patients included in the present study. Outliers in the right-left direction could be due to differences in bladder filling between the patients, causing the bladder to ‘flop’ laterally over the tandem. Bladder filling is not part of the treatment protocol within the department, but can have an impact on the bladder position relative to the cervix. Umesh Mahantshetty et al. evaluated specific bladder filling protocols, including empty, 50 ml and 100 ml bladder volumes, finding each protocol to provide a stable treatment, with no impact on DVH parameters. This ensures that bladder filling between planning and treatment remains consistent, demonstrating the importance of implementing a bladder filling protocol. A center of volume displacement within a 5 mm radius occurs when considering various degrees of bladder filling [20]. Difference in the bladder filling between treatment planning and delivery could affect the treatment plan robustness. Without a consistent bladder filling protocol, patients may be impacted by dwell position uncertainty to varying degrees.
There is a patient-to-patient variation in treatment plan robustness due to the individual anatomy and treatment needs, causing the treatment plan to be either more or less sensitive to dwell position displacement. If the individual treatment plan contains dwell positions that are near to a critical volume edge, the displaced dwell position(s) will enter or exit the volume, leading to a large change in dose. During optimization of the treatment plan, dwell positions are weighted to accomplish a conformal plan for the predetermined treatment endpoints. When the plans are heavily optimized, a few dwell positions contribute substantially to the total dose [14]. A displacement of a heavily weighted dwell position can cause a large change in the dose to critical volumes [21]. When planning, evaluation of the treatment plan robustness may be considered using the metric developed by Poder et al., where dwell location and relative weighting is taken into account [22]. Further investigation is required to verify the robustness parameter's application for gynecological application. Differences in dwell weighting combined with anatomical diversity produce a variation in the treatment plan robustness.
A directional dependence in sensitivity to dwell position displacement is clear, with the shifts along the anterior-superior direction having the highest impact on the bladder and rectal doses as well as the HR-CTV coverage. A 2 mm threshold along this axis for organs at risk demonstrates both the treatment and monitoring accuracy required. Sensitivity along each axis can be used to refine quality control of applicators and imaging procedures, or protocols to limit applicator displacement during patient transport and movement on to imaging apparatus in the MRI-CT workflow. For example, implementing a means for needle positions relative to anatomy may be confirmed within 2 mm immediately prior to treatment.
The results of this study coincide with the literature, as Tanderup et al. found a 10-12% increase in the dose to the bladder and rectum for a 2 mm shift in the anterior-posterior direction. The study, which focuses on reconstruction uncertainties, revealed a 12% change in the dose for OAR when considering a ±3 mm shift in all other directions. Tanderup et al. found applicator and needle displacement in the inferior direction, and demonstrated a threshold of 4 mm for the bladder in interstitial cases and 6 mm in intra-cavitary cases [4]. The increased sensitivity when compared to the findings listed in Table 1, could be attributed to the study only evaluating a 3 mm and 5 mm shifts, and assuming a linear relationship between OARs dose and applicator translation.
Table 1
In vivo source tracking error thresholds in millimeters for intra-cavitary and interstitial cases. A negative (–) value signifies an anterior shift when considering the anterior-posterior axes, inferior shift for the superior-inferior axes, and right for the right-left direction. In bold is the in vivo source tracking error threshold in each direction, which achieves both local control and low-risk of complication for each treatment type
Schindel et al. conducted an applicator displacement and reconstruction error study for MRI-guided intra-cavitary brachytherapy, and implemented superior-inferior applicator displacements and dwell position displacement along the applicator channel path. The study found a ±3 mm shift in the superior-inferior direction, which caused a change in rectum D2cc that reached the 10% threshold. Additionally, an inferior shift of 7.5 mm and superior shift of 6 mm caused a change in bladder D2cc of 11 ±5% and 10 ±7%, respectively. These results are generally more sensitive to dwell position displacement than what was found within the present study, with only the change in bladder dose in response to superior displacement coinciding. The data set was analyzed by finding an average impact over 20 patients. As demonstrated in Figure 3, there is a large variation in treatment plans from patient-to-patient. Determining the impact of displacement based on mean value can allow outliers and a minority of heavily optimized treatment plans to influence the results [3].
Organs at risk, i.e., bladder and rectal doses were affected the most in the anterior-posterior direction, with a 2 mm in vivo source tracking error threshold. Local control was affected by a shift in any direction for both interstitial and intra-cavitary cases, the most sensitive of which being a posterior displacement limit of 3 mm for interstitial plans. Applicator rotation was investigated as a possible clinical failure. Minimal change in dose was found within the ±20° investigated, with HR-CTV coverage un-affected and a 20° rotation required to evoke a 10% increase to bladder and rectal doses. These results, presented as a displacement value, along each axis provides data, which can be used to establish limitations for novel treatment monitoring and quality assurance devices. Currently, the literature has a specific focus on determining the dosimetric impact of reconstruction and co-registration uncertainties given the introduction of 3D image-based gynecological brachytherapy. These studies focus on dwell position displacement within a small range, for selected directions, or along the source path. The impending emergence of in vivo source tracking for real-time treatment monitoring means that a comprehensive evaluation of dwell position displacement in all directions is necessary to determine the required accuracy for new technologies or informed action thresholds.
The primary limitation of the study is a simplification of possible dwell position displacement, as it does not account for off-axis shifts or all locations the applicator might take during displacement. Dwell positions are displaced in unison along each axis, which does not simulate all random errors that result in a single catheter shift. Some of these errors include catheter reconstruction error or catheter swap errors. The shift of a highly weighted catheter within each treatment plan could be used to develop a source tracking error threshold, since it would have the largest impact. Additionally, the study does not account for changes in shape or size of OARs due to rectal or bladder filling. The evaluation of applicator rotation serves as an example of a specific clinical scenario assessed for quality assurance. Utilizing a script to change dwell positions in a specific fashion that simulates a treatment scenario can serve as an archetype to test the influence of a multitude of different situations, which are more complicated than displacing the entire treatment plan along one axis. An example being an assessment of interstitial needle bending due to oedema by adjusting dwell positions to sit along a bent path.
In the current study, source tracking thresholds were established for both interstitial and intra-cavitary cases; however, the results demonstrate that the action level of a 10% change in the dose for 25% of the cohort might not be applicable on an individual basis, given a large variation in treatment robustness. Individual cases responding in different capacities following an implemented shift were demonstrated by a growth in inter-quartile range with a displacement.
The study has set out to determine a comprehensive in vivo source tracking error threshold and to validate its’ application in HDR brachytherapy for cervical cancer. Ultimately, it is apparent that an in vivo source tracking error threshold would be patient-specific, as the individual treatment plan optimization and anatomy would dictate a particular tolerance. A combination of the demonstrated script-based methodology and an in-built dose calculation to replace RadCalc in the workflow, would allow automation of the in vivo source tracking error threshold calculation. This would facilitate a practical clinical application of an individualized error threshold for real-time treatment monitoring.
The use of an in vivo source tracking error threshold requires adequate source tracking relative to patient anatomy. It is imperative that source tracking technology is applied in conjunction with pre-treatment imaging to address the movement of anatomy and implant position.
Application of the data derived within this study can provide a dosimetric evaluation for developments in gynecological brachytherapy for cervical cancer. Laura van Heerden et al. assessed the accuracy of dwell position tracking with an electro-magnetic tracking (EMT) brachytherapy system. For cervical treatments, a maximum deviation of 2.4 mm was found for an intra-cavitary setup, while the accuracy in each direction was determined for phantom measurements in a clinical environment [23]. Additionally, an optical-based real-time applicator position monitoring system (RAPS) has also been developed and undergone clinical trials [24]. Both EMT and RAPS-based studies evaluate the respective system ability to determine dwell position along each axis. Data within this study were produced along each axis, and is readily available for application to inform the clinical requirements for localization accuracy of new technologies. Furthermore, the real-time applicator position monitoring system (RAPS) can also use an in vivo source tracking error threshold as an action level. Combination of the RAPS and calculating patient-specific action levels with the methodology established within the current study would prevent overdosing of organs at risk and inadequate HR-CTV coverage.
Conclusions
Determining the dosimetric impact of applicator or dwell position displacement provides a clinical benchmark for the development of pre-treatment verification devices and an action level for real-time treatment monitoring. It was established that an in vivo source tracking error threshold needs to be patient-specific and calculated for each treatment plan, given the fractionated nature of HDR brachytherapy of cervical cancer treatment.